Amphibians
From Comparative Physiology of Vision
Amphibians | |
---|---|
![]() |
|
Tiger Salamander, a commonly studied amphibian species | |
Scientific classification | |
Kingdom: | Animalia |
Phylum: | Chordata |
Class: | Amphibia |
Summary of Amphibian Vision
Amphibians are a class of animals that include three orders: Anura (includes frogs and toads), Caudata (salamanders), and Gymnophiona (caecilians – underground dwelling legless amphibians). [1] These orders exhibit a variety of different characteristics with respect to vision; and some orders have been studied more extensively than others. For example, frogs such as the Leopard Frog (Rana pipiens); Bullfrog (Rana catesbeiana); and the Common Toad (Bufo bufo) have been used as model organisms for the study of the visual system for over 70 years[26]. Other well-studied Amphibians include: the Tiger Salamander (Ambystoma tigrinum); and Old World newts (Triturus) In contrast, very little is known about the Caecilians. [2]
Over 65 million years, the amphibian eye diverged very little from that of fish, with the same inflexible lens that accommodates by moving forward or backward within the eyeball. [3] The transparent nictitating membrane (an apparent advantage for aquatic creatures) was also retained in amphibians. Amphibians have eyes which are adjusted to seeing both on land and in water.
Amphibians are unique in that they are capable of extra-ocular vision - sensing light energy using structures other than the eyes. [1][4] For example, amphibian skin acts as a sense organ which often replaces the need for vision. Light sensitivity in the amphibian's eye depends on the extent of diurnal, crepuscular, nocturnal, and/or fossorial behavior of any given species. [2] Some species, such as the Texas Blind Salamander, dwell underground or in caves and have thus evolved to not have eyes since they live in complete darkness. [5] In some cases, juvenile forms have eyes but these structures degenerate during metamorphosis, the process by which the juvenile adopts its adult form.[6]Caecilians, which burrow and are crepuscular, or twilight-active creatures, have a reduced eye structure and some are blind, or possibly detect changes solely in light intensity.[7][8]
Amphibians have no foveas (areas of highest visual acuity), and the amphibian visual field has high sensitivity and low acuity. They can detect minute changes, but often have difficulty trying to observe a scene in stasis. [9]
Most Amphibians are tetrachromats - their retinas contain 4 types of photoreceptors and they are therefore capable of color vision with a broader sensitivity to color than humans. Two unique photoreceptors exist in amphibians -- green rods that absorb the "blue" wavelength, [2] and a type of rod that contains the purple-receptive porphyropsin, which enables them to see in the UV range. This type of rod is most common in larval amphibians and in species that remain aquatic throughout life, such as salamanders. [1]
Anatomy of the Amphibian Eye
Because anatomy differs widely amongst amphibians in aquatic habitats and those that inhabit terrestrial environments, as well as at differing stages of development, broad generalizations in either anatomy or physiology are not entirely useful, but in some circumstances are necessary due to the lack of species-specific research or access to credible information.
Interestingly, amphibians and particularly salamanders (urodeles) have an enormous capacity for regenerating body parts. This extends to ocular structures as well as limbs. Amphibians are capable of regenerating the entire lens [10] and retina [1], and possibly other ocular structures as well.
Eye Placement
Eye placement is adaptive with regards to environment - as human eyes are situated at the front of the head in order to allow us to see where we are going, most amphibians are subterrestrial or lie solely in aquatic habitats, so their eyes are situated on the top of their head, which allows them to immerse their body in water while keeping their eyes above the water surface. [11]
Eye Size and Shape; Eyelids, Protective Functions, and Glands
Aquatic anurans and all larval anurans lack eyelids, while conversely terrestrial adult anurans have short lids and possess a translucent and elastic nictitating membrane, or third eyelid, which functions for protection of the eye. Another protective function of the eye is the ability of the retractor bulbi muscle to pull the globe, which is nearly spherical in all amphibians, back into the orbit and the levator bulbi to return it to its normal position. This is important in swallowing because only a thin membrane separates the orbit and the mouth. Beyond these two movements, no other globe movement is possible because the six remaining extraocular muscles are vestigial. Urodeles, or salamanders, have well-developed and movable eyelids. Aquatic forms have only primordial lids, while subterranean forms typically possess rudimentary eyes covered by skin. Among these, salamanders that live in dark environments have small, degenerate eyes. The eye is lubricated by Harderian glands of the nictitating membrane and marginal eyelid glands; present in Anurans and Caecilians. Terrestrial salamanders also have lacrimal glands that are distributed along the lower eyelid.[1] "A quiescent frog will close its eyelids synchronously every 0.5 to 5 minutes. They will also be closed by eye irritation, whole body vibration, sudden bright light, large approaching dark objects, and prey swallowing."[12]
The Lens
The lens serves to focus light onto the retina (accommodation) and is rounded to varying extents in vertebrates. In amphibians it is thick and is almost spherical - more so in the tadpole than in the adult, and accomodation is performed through a forward movement of the lens by the protractor lentis muscle, which moves the lens along the optic axis of the eye toward or from the retina. Urodela (salamanders) have only one muscle which acts on the lens, while the Anurans posses two. [7]
The Retina
The retina is the innermost tissue layer of the eye and contains photoreceptors and neurons onto which an image is projected. The amphibian retina is avascular; its blood supply is derived from a membrane on the retinal surface of the vitreous body. Single cones exist as well as photoreceptors representing fused rods and cones that contain colored oil droplets. [7]
The Pupil
The pupil is the region upon the lens which is unobscured by supporting structures, and therefore receptive to light. The amphibian pupil is circular when dilated, but upon constriction can take on several forms depending on the species, ranging from round to ovular but also including some rather irregular shapes such as pear-shaped or heart-shaped, as is observed in the fire-bellied toad. Pupillary light reflexes are controlled consciously and are therefore not a good measure of light response like they are in mammals. [7]
The Sclera
The sclera is the tough, outer casing of connective tissue that forms the globe structure of the eye. The sclera of the adult Anuran contains a posterior cup of hyaline cartilage and, in some species, an anterior ring of bone. The sclera of the Urodele is fibrous and contains neither cartilage nor bone. [7]
The Cornea
The cornea is the transparent portion of the sclera that allows light to enter the pupil. The terrestrial amphibian cornea acts as a dioptric focusing structure and is anatomically characteristic of all vertebrates. The cornea of the larval Anuran is similar to those of fish, in which there are an inner cornea and a second, outer cornea, which fuse at metamorphosis. Though its significance is yet unclear, the cornea of the two Asian giant salamanders is highly irregular in that it possesses blood vessels (vascularization) within its stromal connective tissues. [7]
The Iris
The iris of amphibians is vascularized and is pigmented with melanophores (cells containing melanin and usually present a black or dark brown color), carotenoids, and guanine crystals. [7]
Evolutionary Significance / Unique Visual Optics
There is somewhat of a continuum of eye design characteristics across the vertebrates (animals with backbones). Lampreys (evolutionarily old type of vertebrates) have a lens that is not connected to the eyeball, it simply floats sandwiched between the cornea and the vitreous humor. Accommodation (focusing the image) is performed by extra-ocular (outside the eyeball) muscles, which deform the cornea. In fish, the lens is almost spherical to compensate for the lack of refraction in the cornea. The fish lens is attached to the eye interior by membranes and muscles, which move the lens forward or backward. Because the refractive indices of water and the liquid aqueous humor (which supports the cornea) are so similar, there is little refraction of light in the fish cornea. The spherical lens is thick and thus achieves sufficient refraction of light to focus an “image” on the retina (light sensitive interior surface) of the eye. [13]
Evolutionary History of Amphibians
The first amphibians arose 355 mya, in the early Carboniferous period. The amphibian lineage diverged from that of fish 420 mya, toward the end of the Silurian period [14]. Over 65 million years, the eye structure did not change much; the amphibian eye diverged very little from that of fish, with the same inflexible lens that accommodates by moving forward or backward within the eyeball.[3] The transparent nictitating membrane (an apparent advantage for aquatic creatures) was also retained in amphibians.[15]
Within the class Amphibia there are variations of eye anatomy:
- Order Anura (frogs and toads) show rudimentary ciliary bodies, from which 2 muscles attach to the lens.[13] Most Anurans have good eyesight, on which they depend to hunt their small moving insect prey. [16] They have the biggest and most complex eyes of the Amphibians.[17] Anurans have adapted various methods of utilizing their superior (compared to that of the other amphibians) vision, and are able to hunt a wide variety of prey. [18]
- Order Urodela (salamanders) have only one muscle that moves the lens.[13] They have smaller, more primitive eyes; members of Urodela rely on scent and vision for hunting and mating signals.[19] Urodeles are slow compared to Anurans, and their activities (especially in the more terrestrial species) are mostly nocturnal.[20]
- Apodans mostly live underground and hunt slower prey such as earthworms.[20] Their eyes are degenerated (have evolved to become less complex) to varying degrees, with the most degenerate being completely blind. [2] On the evolutionarily newer side of Amphibia are Reptilia (includes birds) and Mammalia. Members of both classes have ciliary bodies from which either pads or ligaments (zonules) connect to the lens, and can modify lens shape for accommodation. [13] This continuum suggests that a flexible lens (with muscles and/ or ligaments that may change the lens shape) is an advantageous adaptation for primarily terrestrial creatures. In the case of amphibians it appears that, as amphibian ancestors began partially residing on dry land, natural selection drove toward evolution of a dynamic lens; yet evolution of the lens arrested once the class established its niche. The resulting amphibian eye is between that of fish and terrestrial animals, an appropriate structure given the dichotomous nature of the amphibian lifestyle. There are various characteristics of the “camera” style eye that are shared by most vertebrate eyes.
Unique Characteristics
There are a few characteristics that are unique to amphibian eyes. As in Reptiles and Mammals, Amphibian lenses are suspended by zonules (tiny ligaments) that attach to the ciliary bodies. In Reptiles and Mammals, ciliary muscles contained within the ciliary bodies serve to change the lens shape for accommodation.[21] This contrasts with Amphibian accommodation, wherein the lenses are moved forward or backward by pupillary nodules (pads touching the lens). The pupillary nodules are moved by lens muscles located near the ciliary bodies.[22] Anurans possess two pupillary nodules (and two lens muscles), while Urodeles and Apodans possess only one lower pupillary nodule (and lens muscle).[13] Another observed feature unique to amphibians is the choroidal hyaline cartilage: a cartilage that contains lots of collagen and no nerves or vessels. Portions of hyaline cartilage may or may not be covered with perichondrium: a fibrous membrane containing vasculature that can provide nutrition to associated tissues.[23] This choroidal cartilage (formerly known as sclerotic cartilage) is located between the choroid and the retina.[24] “On the retinal surface, there is no perichondrium, and the pigmented epithelium exhibits an intimate relationship to the cartilage layer.”[24] The great prevalence of Anurans (compared with other amphibians) has led to a bias in the body of literature regarding amphibian physiology. Most of what is known is based on Anuran studies. Particularly regarding vision, (the sense in which Anurans are significantly more advanced than their amphibian brethren), more studies are available on Anurans than on other amphibians. The article from which the choroidal cartilage information is derived was performed on two Anuran species; the article cites evidence for similar structures in some salamanders (urodeles). Given the somewhat squishy body of anurans, and the relatively exposed location of their eyeballs; it is logical to presume that this cartilage functions to maintain the structure of the eyeball. Eyeball integrity maintenance is particularly important given the range of physiological processes (aside from hunting and predator avoidance) that anuran eyeballs are involved in: swallowing, vocalization, breathing.[24]
Life Cycle
Anatomical Changes
Most amphibians undergo metamorphosis – the process by which they change from a juvenile, aquatic form to an adult, terrestrial form. During this period of rapid change the eye's primary challenge is to change so that it is useful for vision in air rather than water. During this time the lens changes from nearly spherical, similar to fish; to a flatter shape, like terrestrial mammals. Among Anurans, the tadpole's vision becomes increasingly myopic as it undergoes metamorphosis. Its vision deterioates as the lens becomes flatter, until the tadpole finally moves onto land, where the lens reaches its final, flat state and moves farther from the cornea.[25] The pupil becomes less protuberant. The eyelids, absent in tadpoles, develop.[1] Also, the axis of the visual field changes so that the center is more binocular, and the field of view is more constricted.
Biochemical Changes
Salamanders, newts and frogs utilize different forms of retinal, based on different forms of vitamin A. Vitamin A1 when metabolized produces retinine1 which when associated with the opsin protein produces the pigment rhodopsin, the pigment common in terrestrial vertebrates. Vitamin A2 becomes retinine2, which differs from retinine1 in having an additional double bond in the hexene ring. Reteinine2 in conjugation with the opsin protein is known as the pigment porphyropsin, and is most common in freshwater fish (Fite). In general terrestrial amphibians utilize rhodopsin, while aquatics and larva/tadpoles posses porphyropsin.Tadpoles are more sensitive to green light, while adult frogs tend to be sensitive to blue light. This is presumably because of how the opsin holds the different retinal confirmers (Moore).
The pigment molecule doesn't necessarily stay constant once an amphibian has metamorphosed. For example during mating season newts resume the spectral sensitivity of larvae, since they return to the water, and re-obtain their normal spectra when mating season ends (Fite). The same opsin proteins are produced through out the newts life, however; the different retinal confirmers alter the sensitivity of the protein to make it more responsive to shorter wavelengths, as shorter wavelengths penetrate water further.
The possible evolutionary significance of the different visual pigments is that it could be one of (many) necessary adaptations for life to escape its watery confines. For example the toad Xenopus laevis as an aquatic toad posses primarily porphyropsin as its visual pigment, with a small amount of rhodopsin present, one reason Xenopus laevis may resemble a transitional amphibian.
Visual Field
There exists great variation in the visual capabilities of amphibians. Frogs eyes are well-developed, large, bulging, and can see in virtually all directions at once, giving them the most expansive visual field among vertebrates, with the possible exception of a few fish. Many have good depth and color perception and great acuity for detecting both small and large prey at a close range. They are also skilled at visually detecting distant moving objects. Other Anurans such as most toads and salamanders have eyes better adapted for night or dim-light vision, though many still have the ability to see quite well in lighted surroundings. [2]
The eyes of most larval amphibians are located toward the top or at the sides of the head, but during metamorphosis the eyes move forward, likely due to the nearly-universal feeding upon small animal prey in postmetamorphic individuals. The then-overlapping visual fields of the two eyes contributes to a stronger depth perception for targeting prey. Such binocular vision culminates in lungless salamanders (family Plethodontidae). [2]
Light Sensitivity
There is a broad range in spectral sensitivity that depends on the extent of diurnal, crepuscular, nocturnal, and/or fossorial behavior. Diurnality describes behavior characterized by activity during daylight, crepuscularity by activity during twilight hours (matutinal organisms are specifically active at dawn, and vespertine organisms at dusk), nocturality by nighttime activity, and fossorial behavior by activity of organisms that are adapted to life underground.
Light-intensity preferences, daily activity rhythms, and choice of habitat all influence the often relatively narrow range of light levels during the day at which an amphibian is active. This is the range within which its eyes are best adapted, the optimum ambient illumination (OAI) level for any given species. The biomodal activity pattern of some species may reflect such adaptation. [2]
Motion Detection
Amphibians have no foveas (areas of highest visual acuity) in their eyes.[26] This means that the amphibian visual field has high sensitivity and low acuity. In other words: they can detect minute changes, but often have difficulty trying to observe a scene in stasis. “[A frog’s] eyes do not move, as do ours, to follow prey, attend suspicious events, or search for things of interest. If his body changes its position with respect to gravity or the whole visual world is rotated about him, then he shows compensatory eye movements.”[26] It is notable that the amphibian eyes are not adapted to isolate a specific prey and to focus it’s efforts on that one morsel; amphibian eyes are adapted to detect and rapidly respond to as many changes in scenery as possible, and to lose interest in static objects. This strategy seems congruent with the large populations on which amphibians prey – populations in which any prey target is as good as the next.
It seems a general theme that rods have much greater convergence than cones.[27] Presumably, amphibians have a much higher percentage of rods than cones, and thus their populations of photoreceptors are very highly converged. This means that for each ganglion cell, there are multiple bipolar cells, and 100s or 1000s of photoreceptors. Thus, the only way for an amphibian to perceive an object, shadow, etc., is if it is moving across the visual field. When the scenery is static, there isn’t enough info coming to any one ganglion cell to produce any meaningful alteration of AP frequency. For example, if a frog possessed the mental ability to read, it could only do so if the words were continually passed before it's eyes. Even then, his visual acuity might not be sufficient to distinguish letters; but if he tried to read a stationary page, it would just appear a gray blur.
When you see something in peripheral vision, attention is drawn. You may know that something has moved, but not exactly what. Imagine that your whole visual field is like the peripheral, and that you automatically lunge toward and attempt to eat any moving object that seems a lot smaller than you and that moves erratically enough to seem lively. Imagine that anything static (not moving) in your visual field blurs and fades to obscurity. This is what it would be like if you were an amphibian.
Various data show that many amphibians are able to judge distances, some with binocular vision and others with monocular vision. “Plethodontid salamanders are the only amphibians with forward looking eyes and binocular vision that closely parallels the well developed binocular vision of many reptiles, birds, and mammals.”[2] Yet some Anurans also have enough overlap of their right and left visual fields to significantly enhance their ability to accurately judge the distance to their prey. However, many monocular techniques such as motion parallax and kinetic depth perception confer depth perception abilities on all the other amphibians.[28]
- Motion parallax: As the observer moves, the apparent movement of stationary objects against the background gives clues to their relative distance away.[29]
- Kinetic depth perception: as objects move closer or further away from the observer, they appear to grow or shrink in size. This provides depth information about moving objects.[29]
Color Vision
Some amphibians are supposedly dichromatic (using two types of photoreceptors for a limited sense of color), however many species are trichromatic or tetrachromats - capable of color vision with a broader sensitivity to color than humans owing to four types of photoreceptors. The “color” (wavelength of light) that a photoreceptor cell is optimally responsive to is determined by the type of opsin proteins contained in that cell’s disk epithelia.[30]
Adult amphibians typically have 4 types of photoreceptors:[2]
- 2 types of rods:
- "Red" Rods: Common to all vertebrates, and contain rhodopsin, which has peak absorbance (greatest likelihood of being activated) at 502 nm (wavelength), which is the green wavelength.[2]
- "Green" Rods: Unique to amphibians, and have peak absorbance at 433 nm: the blue wavelength.[2]
- Some amphibians possess a third type of rod photoreceptor that contains the purple-receptive porphyropsin. Porphyropsin is most commonly found in larval amphibians and in species that remain aquatic throughout life.[31] This rod ennables them to see in the ultraviolet range. They use this special vision adaptation to hunt prey. Perception of UV light is adaptive for amphibians because the higher energy wavelengths are more likely to travel through the dense medium of water and reach the organism.
- 2 types of cones:
The functional differences between photoreceptor types are caused by the presence of different opsin proteins in the stacks of membranous disks within each photoreceptor cell. Each different opsin molecule can hold one (much smaller) molecule of retinal. The structure of retinal allows its shape to be changed by an incoming photon (wave) of light. The likelihood that a given photon will do this is increased by the orientation in which the opsin holds its retinal.
The species Rana catesbeiana is known to have up to 40% porphyropsin concentrated in the upper third of the retina. It is an amusing adaptation to enhance the species’ behavior of sitting with half of its eyes above water, and half below. The refractive nature of the lens causes underwater light to hit the upper retina, and above water light to hit the lower retina.[31] The color receptive photoreceptors are also arranged in somewhat circular arrangements, with different colors in the center and surround. Consider a blue center, green surround receptive field. If blue light shines on center, bipolar cell is excited. If green light shines on surround, bipolar cell is inhibited. If either diffuse blue or diffuse green shines on the whole receptive field, bipolar cell is neither excited nor inhibited. If blue shines on center and green shines on surround, bipolar cell is moderately excited. If green shines on center and blue shines on surround, bipolar cell is moderately inhibited.
Phototransduction and Retinal Processes
In amphibian eye phototransduction, "the retina is connected to the optic tectum by at least three types of ganglion cells, each with an excitatory receptive field and a surrounding inhibitory receptive field, but they differ in the diameter of their central excitatory receptive fields. Diameters in Class 2 ganglion cells are approximately four degrees visual angle. Those in Class 3 cells are about eight degrees and Class 4 ganglion cells range from twelve to fifteen degrees. As stimuli move across the toad’s visual field, information is sent to the optic tectum in the toad’s midbrain. The optic tectum exists as an ordered localization system, in the form of a topographical map. Each point on the map corresponds to a particular region of the toad’s retina and thus its entire visual field."[32]
The amphibian retina contains 2 functional layers. The pigmented epithelium (pars pigmentosa) is the outermost part of the retina, and mostly contains the photoreceptors (rods and cones). On one end, each photoreceptor is embedded in the pigmented epithelium. On the other end, photoreceptors synapse with neurons (bipolar cells and horizontal cells), and the bipolar cells synapse with more neurons (amacrine cells and ganglion cells).[33] This inner, neuronal layer is the pars nervosa or optica).[24] There is significant convergence from the population of photoreceptor cells to the ganglion cells (which transmit visual information to the brain).[34] The amount of convergence varies with location on the retina. A larger receptive field (greater convergence of many photoreceptors to few ganglion cells) produces a blurrier image because “the larger [receptive] field provides less information about exactly where light struck the retina.”[35]
An analogy for convergence
The president of a company wants to know the status of his employees – happy or sad. One manager manages 500 employees. All 500 shout to him at once their emotional status, and the manager must integrate all 500 shouts into one status. He thinks he hears more people shout “happy” than “sad”, so he reports happy employees to the president. The president has very little information about each individual employee, just an overview of the majority. Another manager manages only 3 employees, 2 of which shout “sad” and 1 of which shouts “happy.” This second manager reports sad employees to the president. The president has accurate information about 2 out of 3 individuals. In this analogy the president is the brain, the managers are the ganglion cells, and the employees are the photoreceptor cells. It is the brain that “sees” by interpreting the data represented by the variety of signals being generated in the retina. A retina disconnected from a brain will “see” absolutely nothing.
Phototransduction
Transduction is the translation of a stimulus from the external environment into an internal signal, which is interpreted by the brain. Phototransduction is the transduction of light entering the eye. The complexity of the eye determines what level of detailed information can be recieved, and thus how useful vision is to the organism. Most amphibians have somewhat complex eyes (compared with the rest of the animal kingdom), and vision is useful (in the case of Urodeles and Apodans), and is the primary (for Anurans) sense used to gather information from their surroundings.
Campbell[9] gives an overview of phototransduction in the vertebrate retina. We will discuss a generalized photoreceptor; whether rod or cone, the chemical transduction cascade is generally similar. Each ganglion cell has a “baseline” frequency at which it fires action potentials. Visual perception is based on the ability of a photoreceptor to change this action potential frequency in response to varying degrees of light or darkness. For example, in a featureless gray room, all the ganglion cells would be firing at their baseline frequency. Any deviation from gray will be detected by the photoreceptors and transduced into either an increase or decrease in the action potential frequency of the ganglion cells. This change is interpreted by the brain as either light or dark.
Here is an outline of phototransduction:
- A photon of light hits retinal (contained within opsin), and changes its shape. The retinal is still connected to the interior of the opsin, so its shape change induces a subsequent shape change in the opsin molecule.
- This activated opsin activates another protein - Transducin (a G protein)
- Activated transducin attaches to the enzyme Phosphodiesterase (PDE), causing it to become active.
- Acitvated PDE performs chemistry, which causes open sodium channels to close.
- In this state, potassium is flowing out of the cell, and sodium can no longer flow in. Potassium and sodium ions are both positive, so the result is that the overall charge inside the cell becomes more negative (hyperpolarization). This change in electrical potential (charge) within the photoreceptor cell is a receptor potential.
- The receptor potential causes voltage gated calcium channels to close. Voltage gated channels are those whose opening or closing is caused by electrical potential changes within the cell. The influx of calcium that is required for continual glutamate release is halted; and glutamate release slows. (Glutamate is both an amino acid, and a neurotransmitter. A neurotransmitter is a chemical that either increases or decreses the AP frequency of neurons that are receptive to that neurotransmitter. The “preferred” state of photoreceptors is darkness. In darkness, the photoreceptors tonically (constantly) release glutamate. Light inhibits this tonic release of glutamate, and thus alters the AP frequency of associated ganglion cells. The chemical processes by which said inhibition occurs require more energy that those processes which maintain the baseline AP frequency. Thus, darkness is said to be the preferred state.)
- The chemical signal of glutamate is received by the horizontal cells and the bipolar cells. This is the first location of lateral inhibition: when a horizontal cell is stimulated by an activated photoreceptor, the horizontal cell inhibits distant photoreceptors and bipolar cells that are not active. The effect is that the light spot appears lighter (than it would without lateral inhibition) and the surroundings appear darker.
- While horizontal cells synapse only with photoreceptors and bipolar cells; the bipolar cells continue the pathway to the brain by synapsing with ganglion cells and amacrine cells. Amacrine cells perform lateral inhibition similar to that of the horizontal cells.
- Only ganglion cells have the protruding axons that form the optic nerve fibers. “The right and left optic nerves meet at the optic chiasm near the center of the base of the cerebral cortex.”[36] The optic nerves project (connect) to the right and left lateral geniculate nuclei of the thalamus. However, after the optic chiasm the optic fibers diverge, so that each of the lateral geniculate nuclei receives information from both eyes. This organization forms the basis for binocular (3 dimensional) vision.
- Neurons from the lateral geniculate nuclei project to the primary visual cortex in the occipital lobe of the cerebrum, and elsewhere in the brain. Probably 30% of the whole cortex takes part in interpretation of visual data. The amount of brain involved with visual perception is a testament to its importance as a sense.
To understand phototransduction, one must remember the high degree of convergence over most of the retina. The convergence we see in the retina of photoreceptors on bipolar cells and ultimately on ganglion cells is the first step in processing of visual stimuli. The cells that ultimately converge on ganglion cells are the cell's receptor field, and their response to stimuli is what ultimately leads to the frog’s perception.
Classes of amphibian ganglion cells
It was experimentally determined as early as the 1960’s that frogs can have 5 classes of ganglion cells:
Class 1: Sustained Edge Detection
Class 1 receptors have oval receptive fields from 1.5 to 4 degrees in size. These cells respond maximally to moving or stationary edges of light or dark objects, and detect the completeness and sharpness of an edge, including that from a spot which is either brighter or darker than its background. "If a boundary is brought into [the toad's] receptive field in total darkness and the light switched on, a continuing response occurs after an initial delay. The response is enhanced by edge movement. If an edge stops in its visual field, the neuron remains activated for several minutes. In this case, if the light is turned off, the response ceases only to reappear less intensely when the light is turned on again."[12]
Class 2: Convex Edge Detection
Class 2 receptors have oval receptive fields ranging in size from 2.5 to 5 degrees. They are responsible for the detection of the dark leading edge (head) of any worm or bug. "If the stimulus stops before the center of the receptive field, the neuron exhibits a slow decaying response (time constant between 0.3 to 3 seconds)."[12] They are similar to Class 1 cells, however unlike the Class 1 neuron a brief darkness or shadow will permanently stop the response until the object moves again, and they cannot detect diffuse light. These types of neurons have a strong inhibitory surround region indicating that they are prevented from working in environments with substantial background clutter.[12]
- Class 1 and 2 cells have inhibitory surrounds (on center/off surrounds), so large dark objects illicit less of a response than small dark objects (Fite). These also enable frogs to distinguish corners. Whether a signal coming from a photoreceptor is inhibitory or excitatory depends on the glutamate receptors on the bipolar cell, for example, the bipolar cells in the ‘off’ surrounds would decrease their action potentials when more glutamate is present. [37]
Class 3: Changing Contrast Detection
"These neurons are also known as on-off cells and they have receptive field sizes between 6 and 10 degrees. The intensity of their response is governed by the amount of light intensity change. This includes such parameters as stimulus size but not shape."[12] These cells respond to both local and diffuse changes in light. They are very sensitive to movement, but do not respond to stationary objects.
Class 4: Dimming Detection
These neurons have oval receptive fields ranging from 10 to 15 degrees. They exhibit a prolonged response to a dimming of light.[12] These responses depend primarily on the size of decreased illumination in the receptive field.
- "The Class 3 and Class 4 ganglion cells project to the brain on myelinated fibers which make up only 3% of the optic nerve. Class 3 fibers have a conduction velocity of between 1 and 5 meters per second, Class 4 have a conduction velocity of 10 meters per second, and a small set of fibers having a conduction velocity of 20 meters per second is thought to arise in the brain and project to the retinal neurons."[12]
Class 5: Tonic Darkness Detection
Though they are few, Class 5 neurons seem to indicate the general light level of the frog’s surroundings. When the light level is reduced these neurons slowly increase their firing rate.[12] These cells are always active, and increase activity as illumination decreases.
For all classes of ganglion neurons, the more rapid the angular velocity of a stimulus, the greater the frequency of the action potentials, but with the consequence of a shorter pulse length. Thus, the total number of impulses probably remains the same.[12]
Neuronal Processing
The optic tectum (aka superior colliculus) is the highest brain center for visual motor control mechanisms.[2] The optic tectum is an omnipresent structure of the vertebrate brain.[38] Its structure is somewhat varied across vertebrates, but the layered structure and input from the optic nerve and other somatosensory nerves are highly conserved characteristics.[38] The optic tectum is part of the midbrain, and serves to integrate various sensory information. In amphibians, the optic tectum appears as prominent optic lobes, and it does most of the processing of visual information.[39] The various optic nerve fibers that come from each ganglion cell are bundled together to form the optic nerve. However, when they innervate the optic tectum, they do so in a way that forms a phototopic map that is organizationally representative of the retina.[26]
Inhibitory thalamic pathways help to distinguish between motion of an object and apparent motion of an object, due to movement of the amphibian itself.[40] It has been demonstrated that the abundant neuropeptide Y (NPY) has an inhibitory modulating effect on the retinorecipient laminae (layers that respond to retinal nerve signals) of the optic tectum in 2 evolutionarily distant Anuran species.[41] NPY isn’t necessarily the primary neuromodulator of the optic tectum, but it appears to be a good candidate.
General Higher-Order Visual Perception
It has been demonstrated that certain retinal neurons are optimally activated by general types of moving objects. For example: something with the size and movement pattern of a bug preferentially activates certain retinal neurons, and illicits behavior from a corresponding class of neurons.[40] However, the response of a single ganglion cell receptive field may be velocity dependent, and is not a fixed characteristic of the ganglion cell. For example, “a single neuron may prefer ‘bugs’ at high velocities and ‘worms’ at lower velocities. Furthermore, the response properties of different neurons change with velocity in different ways.”[42]
Additional evidence for the presence of higher order processing in amphibian vision is illustrated by the following example. “Neither retinal ganglion cells nor visual neurons in the optic tectum are capable of distinguishing between large stimuli that are far away and small stimuli that are closer to the retina.”[42] Without some higher visual processing, nothing would prevent an amphibian from lunging at a cow that is so far off that it appears to be the size of a fly.
The observation that different neurons respond differently to different stimuli, under different conditions, indicates that there must be some higher order processing before the amphibian reacts to a stimulus.[42] It has been determined by making precise lesions in toad brains that the optic tectum is involved in normal prey responses, while the thalamic-pretectum region triggers avoidance behaviors (Fite). An interesting note here is that the thalamic-pretectal region acts as more of an inhibitory processor than a behavior inducer. As toads who have lost function of their optic tectum will regain prey responses if their thalamic-pretectum is eliminated, they also lose all predator/avoidance responses (Fite).
It was demonstrated that salamanders’ response to stationary prey may be modified by experience (the salamanders can learn to attack prey that they wouldn’t normally attack). In the wild, Salamanders have a dual sense hunting strategy: they will sniff out and attack certain types of prey in darkness, but will ignore this same prey in light provided that it is stationary (despite the fact that salamanders can see stationary objects).[43]
In salamanders raised with moving prey, reaction to stationary prey is guided by smell. In salamanders raised with stationary prey, reaction to stationary prey is guided by vision, showing that the salamander significantly adapted from what would have been it's natural predation strategy.[43] Adaptation to this degree indicates that post tectal visual processing is sufficiently complex to enable adaptations via memory and learning.
Regeneration and Plasticity
Amphibians have impressive regenerative properties and amazing plasticity in the neuronal connections throughout their body. Not only do they have the ability to regenerate whole limbs (bone, skeletal muscle, and other tissue) they also have the ability to regenerate neuronal connections. Many species of animals have been known to regenerate nerves in damaged tissue of the body, but amphibians have been found to regenerate neurons in the brain, spinal cord, and large nerve fibers, like the optic nerve. For example, the connections between the eye and optic tectum have been greatly studied in many various amphibia. Orderly mapping by the axons of ganglion cells in the retina, and the spatial arrangement of the retina onto the tectum have been shown to depend upon development and specificities that exist between retinal and tectal neurons. Both vision and topographic relations between retina and optic lobe is restored, and connections reform, when the optic nerve is cut and allowed to regenerate. In contrast to certain amphibians, loss of neurons or interruptions of connective pathways in mammalian visual systems lead to permanent damage and loss of vision.
Amphibians can also regenerate the neural retina and lens of their eye as they do their other tissues. Regeneration of these tissues are possible by dedifferentiation (loss of phenotype specific structure) of differentiated stem cells at the site where injury occured. These cells then redifferentiate in response to signals from neighboring cells (the damaged cells) telling them to differentiate into the cells required for the regeneration of lost tissue.
Another example of plasticity in amphibian vision is their ability to adapt to permanent injuries, in order to correct their eye sight and visual field. For instance, frogs have a 360 degree visual field and have binocular projection of the nasosuperior field onto the optic lobe. This allows each visual location (from both eyes) to evoke responses on the same spot of the tectum through either eye. An experiment was performed in which, early in development, the eye of a frog was rotated or inverted in the orbit of it's eye. After metamorphasis, the ipsilateral projection from the normal eye onto the same optic lobe was mapped electrophysiologically, and it was found to have rotated as well, conforming to the distorted contralateral projection. Commissural neurons form several connections with tectal neurons, from temporal conjunctions with the direct visual projection from the opposite eye, establishing precise binocular correspondence of retinal points. This makes it possible for the animal to discriminate distance and depth by central nervous recognition of image disparity.
Feature Analysis in Toads
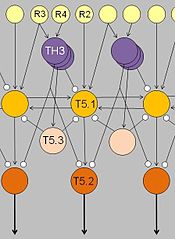
Their response characteristic results from integration in a neuronal network involving retinal gangion cells R2, R3, R4, pretectal thalamic neurons TH3, and tectal neurons T5.1, T5.3.
Arrows: excitatory connections; lines with terminal dots: inhibitory influences.
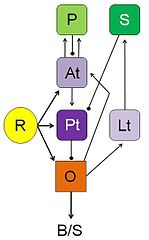
R: retina; O: optic tectum;
Pt: pretectal thalamus; Lt: lateral anterior thalamic nucleus;
At: anterior thalamus; P: posterior ventromedial pallium;
S: caudal ventral striatum; B/S: bulbar/spinal pre-motor/motor nuclei;
arrows: excitatory input;
lines with dots: inhibitory influences.
Ewert studied the hunting behavior of the common toad (Bufo bufo) and observed a natural sequence that consisted of “stalking, binocular fixation, snapping, swallowing and mouth-wiping.”[44][45][46][47] The toad’s actions seemed to be dependent on specific features of the sensory stimulus: whether the stimulus demonstrated worm or anti-worm configurations. “The worm configuration, which signaled prey, was initiated by movement along the object's long axis, whereas anti-worm configuration, which signaled predator, was due to movement along the short axis.[48] He used these observations to develop studies in which electrodes inserted into the frog's brain can measure electrical stimulus. First and foremost, the results allowed him to understand the way the visual system is constructed and connected to the central nervous system. Secondly, he discovered areas of the brain that were responsible for differential analysis of stimuli.
Feature detection is a process by which specialized nerve cells in the brain respond to specific features of a visual stimulus, such as lines, edges, angle, or movement. The nerve cells fire selectively in response to stimuli that have specific characteristics; such as shape, angle, or movement. When the visual information changes, feature detector neurons will quiet down, to be replaced with other more responsive neurons.
Ewert's results determined that there were no “worm-detectors” or “enemy-detectors” at the level of the retina. Instead, he found that "the optic tectum and the thalamic-pretectal region (in the diencephalon) play significant roles in the analysis and interpretation of visual stimuli."[49] Stimulation showed that the tectum initiates orienting and snapping behaviors. It contains many different visually sensitive neurons, among these Type I and Type II neurons (later named T5.1 and T5.2, respectively). Type I neurons are activated when an object traversing the toad’s visual field is extended in the direction of movement; Type II neurons, too, but they will fire less when the object is extended in a direction that is perpendicular to the direction of movement. Those T5.2 neurons display prey-selective properties. The discharge patterns of these neurons – recorded in freely moving toads – “predict” prey-catching reactions, e.g. the tongue flip of snapping. Their axons project down to the bulbar/spinal motor systems, such as the hypoglossal nucleus which harbors the motor neurons of the tongue muscles. In combination with additional projection neurons, prey-selective cells contribute to the ability of the tectum to initiate orienting behavior and snapping, respectively.
The thalamic-pretectal region initiates avoidance behavior in the toad. More specifically, electrical triggering of the thalamic-pretectal region initiates a variety of protective movements such as eyelid closing, ducking and turning away.[44][46] When the thalamic-pretectal region was stimulated, the toad exhibited escape responses, but when the tectum was stimulated in an area close to prey-selective neurons, the toad engaged in prey catching behavior.[50] Various types of neurons in this region are responsible for the avoidance behaviors and they are all sensitive to different types of stimuli. One type of neurons (TH3) is activated by large threatening objects, especially those ones that are extended perpendicularly to the direction of motion. Another type (TH6) is activated by a looming object moving toward the toad. Still other types (TH10) respond to large stationary obstacles, and there are also neurons responding to stimulation of the balance sensors in the toad’s ear. Stimulation of (a combination of) such types of neurons would cause the toad to display different kinds of protective behaviors.
Lesioning experiments led to the discovery of pathways extending between the tectum and the thalamic-pretectal region. When the tectum was removed, orienting behavior disappeared. When the thalamic-pretectal region was removed, avoidance behavior was entirely absent while orienting behavior was enhanced even to predator stimuli. Furthermore, prey-selective properties were impaired both in prey-selective neurons and in prey-catching behavior.[48] Finally, when one half of the thalamic-pretectal region was removed, the disinhibition applied to the entire visual field of the opposite eye. These and other experiments suggest that pathways, involving axons of type TH3 cells, extend from the pretectal thalamus to the tectum, suitable to modulate tectal responses to visual stimuli and to determine prey-selective properties due to inhibitory influences. Ewert and coworkers demonstrated in toads that there are stimulus-response mediating pathways that translate perception (of visual sign stimuli) into action (adequate behavioral responses).[49]
Citations
- ↑ 1.0 1.1 1.2 1.3 1.4 1.5 Duellman, WE. Biology of Amphibians. McGraw-Hill: New York. 1986.
- ↑ 2.00 2.01 2.02 2.03 2.04 2.05 2.06 2.07 2.08 2.09 2.10 2.11 2.12 2.13 Stebbins, Robert C., and Nathan W. Cohen. "Eyes and Vision." A Natural History of Amphibians. Princeton, NJ: Princeton UP, 1997. 42-53. Print.
- ↑ 3.0 3.1 http://www.homeschoolonline.co.uk/biology/vertebrates/vertebrates-introduction-to-the-amphibians.html
- ↑ Adler, Kraig. "The Role of Extraoptic Rhotoreceptors in Amphibian Rhythms and Orientation: A Review." Journal of Herpetology. 4. 3/4(1970):99-112.
- ↑ "Wonderful World of Amphibians and Reptiles." New Ecopsychology. Web. 23 Nov. 2011. <http://www.new-ecopsychology.org/en/amphibia/index-ar.htm>.
- ↑ Sivak, JG and Warburg, MR. "Changes in Optical Properties of the Eye During Metamorphosis of an Anuran, Pelobates syriacus." Journal of Comparative Physiology. 150 (1982): 329-332. Print.
- ↑ 7.0 7.1 7.2 7.3 7.4 7.5 7.6 Williams, David L., and Brent R. Whitaker. "The Amphibian Eye: A Clinical Review." Journal of Zoo and Wildlife Medicine 25.Reptiles and Amphibians (March 1994): 18-28. Print.>
- ↑ Augustine, Ron. "Amphibian Eye Vs. Human Eye." EHow.com. Web. 24 Nov. 2011. <http://www.ehow.com/facts_5643133_amphibian-eye-vs_-human-eye.html>.
- ↑ 9.0 9.1 Biology 7th ed. Campbell and Reece, Pearson Benjamin Cummings
- ↑ Reyer, RW. "Regeneration of the Lens in the Amphibian Eye." The Quarterly Review of Biology. 29. 1(1954): 1-46.
- ↑ Duellman, William Edward, and Linda Trueb. Biology of Amphibians. Baltimore: Johns Hopkins UP, 1994. Print.
- ↑ 12.0 12.1 12.2 12.3 12.4 12.5 12.6 12.7 12.8 Olmsted, David D. "Frog and Toad Eyes." Simple Brain Simulation Resources. 23 Sept. 2006. Web. 29 Nov. 2011. <http://neurocomputing.org/FrogEye.aspx>.
- ↑ 13.0 13.1 13.2 13.3 13.4 http://en.wikipedia.org/wiki/Lens_(anatomy)/>
- ↑ Biology 7th ed. Campbell and Reece, Pearson Benjamin Cummings. P685.
- ↑ http://en.wikipedia.org/wiki/Nictitating_membrane
- ↑ Smithsonian Natural History. Dorling Kindersley, 2010. P367.
- ↑ Structural aspects of an unusual polarized hyaline cartilage in the eyeball of Bufo ictericus and Rana catesbeiana (Amphibia, Anura): morphological and physiological significance. Andrea Souza de Jesus Santana, et al. Micron 36 (2005) 89-93.
- ↑ Smithsonian Natural History. Dorling Kindersley, 2010. P362.
- ↑ Smithsonian Natural History. Dorling Kindersley, 2010. 365-366.
- ↑ 20.0 20.1 Smithsonian Natural History. Dorling Kindersley, 2010. P366.
- ↑ Biology 7th ed. Campbell and Reece, Pearson Benjamin Cummings. P1059.
- ↑ http://www.vetmed.wisc.edu/pbs/dubielzig/pages/coplow/PowerPoints/Wildlife_Dz_Worksh_08.pdf
- ↑ http://en.wikipedia.org/wiki/Hyaline_cartilage
- ↑ 24.0 24.1 24.2 24.3 Structural aspects of an unusual polarized hyaline cartilage in the eyeball of Bufo ictericus and Rana catesbeiana (Amphibia, Anura): morphological and physiological significance. Andrea Souza de Jesus Santana, et al. Micron 36 (2005) 89-93.
- ↑ Sivak, JG and Warburg, MR. "Changes in Optical Properties of the Eye During Metamorphosis of an Anuran, Pelobates syriacus." Journal of Comparative Physiology. 150 (1982): 329-332. Print.
- ↑ 26.0 26.1 26.2 http://jerome.lettvin.info/lettvin/Jerome/WhatTheFrogsEyeTellsTheFrogsBrain.pdf
- ↑ Biology 7th ed. Campbell and Reece, Pearson Benjamin Cummings. P1060.
- ↑ Duellman, WE. Biology of Amphibians. McGraw-Hill: New York. 1986. P383.
- ↑ 29.0 29.1 http://en.wikipedia.org/wiki/Depth_perception
- ↑ Biology 7th ed. Campbell and Reece, Pearson Benjamin Cummings. P1061.
- ↑ 31.0 31.1 Duellman, WE. Biology of Amphibians. McGraw-Hill: New York. 1986. P382.
- ↑ http://en.wikipedia.org/wiki/Vision_in_toads
- ↑ Biology 7th ed. Campbell and Reece, Pearson Benjamin Cummings. P1062.
- ↑ Biology 7th ed. Campbell and Reece, Pearson Benjamin Cummings. P1062.
- ↑ Biology 7th ed. Campbell and Reece, Pearson Benjamin Cummings. P1062.
- ↑ Biology 7th ed. Campbell and Reece, Pearson Benjamin Cummings. P1063.
- ↑ Human Physiology 5th ed. Dee Unglaub Silverthorn. Pearson Benjamin Cummings.
- ↑ 38.0 38.1 http://en.wikipedia.org/wiki/Superior_colliculus
- ↑ Biology 7th ed. Campbell and Reece, Pearson Benjamin Cummings. P1029.
- ↑ 40.0 40.1 Duellman, WE. Biology of Amphibians. McGraw-Hill: New York. 1986. P383.
- ↑ Neuropeptide Y suppresses glucose utilization in the dorsal optic tectum towards visual stimulation in the toad Bombina orientalis: A [14C]2DG study. Simone Funke et al. Neuroscience Letters (2006) 43.
- ↑ 42.0 42.1 42.2 Mechanistic vs. organismal biology. Review of Gerhard Roth’s Visual Behavior in Salamanders. Klisa C. Nishikawa, Herpetologica, December 1989.
- ↑ 43.0 43.1 The Interaction of the Visual and the Olfactory Systems in Guiding Prey Catching Behaviour in Salamandra salamandra (L.) Gisela Luthardt and Gerhard Roth, Behaviour, vol. 83. 1982.
- ↑ 44.0 44.1 Ewert, J.-P. (1974). The neural basis of visually guided behavior. Scientific American, 230(3), 34-42.
- ↑ Ewert, J.-P. (1980). Neuroethology: an introduction to the neurophysiological fundamentals of behavior. Springer-Verlag, Berlin/Heidelberg/New York.
- ↑ 46.0 46.1 Ewert J.-P. (2004) Motion perception shapes the visual world of amphibians. In: Prete F.R. (Ed.) Complex Worlds from Simpler Nervous Systems. Cambridge, MA, MIT Press, pp.117-160.
- ↑ Ewert J.-P., Schwippert W.W. (2006) Modulation of visual perception and action by forebrain structures and their interactions in amphibians. In: Levin E.D. (ed.) Neurotransmitter Interactions and Cognitive Function. Birkhäuser, Basel, pp.99-136.
- ↑ 48.0 48.1 Zupanc, G. (2004) Behavioral Neurobiology: An Integrative Approach. Oxford University Press. 121-132.
- ↑ 49.0 49.1 http://en.wikipedia.org/wiki/Vision_in_toads
- ↑ Carew, T.J (2000).Feature analysis in Toads. In Behavioral Neurobiology, Sunderland, MA: Sinauer, 95-119.
General References
http://www.devbio.biology.gatech.edu/?page_id=893
http://bioportal.bioontology.org/ontologies/46012/?p=terms&conceptid=AAO:0010340
http://universe-review.ca/R10-33-anatomy.htm#amphibians
http://www.ncbi.nlm.nih.gov/pmc/articles/PMC2913695/?tool=pmcentrez
http://www.nlm.nih.gov/medlineplus/ency/article/002319.htm
http://www.ehow.com/facts_5643133_amphibian-eye-vs_-human-eye.html
Miller, JA. "Eye to (Third) Eye." Science News. 128. 19(1985): 298-9. Print.
Reuter, TE et al. "Rhodopsin and Porphyropsin Fields in the Adult Bullfrog Retina."Journal of General Physiology. 58(1971): 351-371. Print.
Reyer, RW. "Regeneration of the Lens in the Amphibian Eye." The Quarterly Review of Biology. 29. 1(1954): 1-46.
Adler, Kraig. "The Role of Extraoptic Rhotoreceptors in Amphibian Rhythms and Orientation: A Review." Journal of Herpetology. 4. 3/4(1970):99-112.
Fite, Katherine. The Amphibia Visual System; a multidisciplinary approach. New York: Academic Press inc, 1976. Print.
Suggested Questions
1. Q: Which is more common among the vertebrates? a. a thick, nearly spherical lens (amphibians) b. a thinner flatter lens (primates)
2. Q: How many classes of ganglion cells does the amphibian eye contain? A: Five
3. Q: What two types of photoreceptors are unique to amphibians? A: Green rods and purple-receptive porphyropsin rods that detect in the UV range
4. Q: Name one anatomical element of the amphibian eye that contributes a protective function. A: Nictitating membrane and the ability of the retractor bulbi muscle to pull the globe back into the orbit
5. What does the retina of the amphibian eye lack, and how is accommodation performed? A: Lack foveas; accommodation is performed through a forward movement of the lens by the protractor lentis muscle, which moves the lens along the optic axis of the eye toward or from the retina.